
-
My research activities address the structure, dynamics, and composition of planetary interiors, and
more specifically of the mantles of rocky planets, including our Earth, and ofthe ice layers of icy
moons and dwarf planets, such as Europa, Titan, and Pluto. These activities imply a multidisciplinary
approach that combines numerical simulations of convection (which is thought to be the main mode of
heat and mass transfer within planetary bodies), mineral physics data (which gives information on the
physical properties of planetary mantles' materials), and of course geophysical observations, when
they are available.
Because convection in planetary mantles may be affected by many different parameters, a central task of my research is to better understand the individual roles played by each of these parameters. For this, I perform numerical simulations with different levels of complexities. Important parameters include the mode of heating (i.e., the source of energy driving convection), the rheology (the way rock or ice are deforming), and the presence of different chemical components with different properties (for instance, different densities and viscosities). I'm more specifically using the code StagYY, developped by Paul Tackley (Institute of Geophysics of ETH Zürich) and collaborators.
In the case of the Earth, different chemical components are likely present in the deep mantle, and may play a key role in explaining today's structure and dynamics. The thermo-chemical structures predicted by simulations can be tested against existing observations to identify the best possible model. Currently, the best constraints on the deep mantle structure are obtained from travel time seismological data, from which tomographic models (i.e., 3D-maps of seismic velocity changes) are built. However, other observables that may provide hints on the structure of the deep mantle. These include the topography of the boundary separating the mantle and the core, seismic attenuation (which can be estimated from seismic waveforms), and seismic normal modes.
In the case of icy moons and dwarf planets, models of convection may be used to reconstruct the thermal histories and radial structures of these bodies, which can be, again, tested against available data. These bodies are typically composed of a rocky core surrounded by layers of ices. Due du the phase diagram of water, a liquid layer, or sub-surface ocean, may exist today in between the core and the ice Ih layer in the case of bodies like Europa and Pluto, or in between a high pressure ice layer and the outer ice Ih layer in the case of larger bodies (Titan, Ganymede). Several parameters may influence the cooling of these bodies. In particular, heating due to tidal dissipation, if present may play a key role. More details on these activities can be found below (numbers in brackets refer to publications listed here).
Structure of the upper mantle and deformation of the lower crust
• Thermo-chemical structure of the upper mantle. A source of seismic heterogeneity in the upper mantle is the ocean/continent dichotomy and, within continents, the presence of cratonic roots, which may extend as deep as 300 km. To better constrain the structure of the upper mantle, I developed methods based on the modelling of gravity anomalies to constrain density distributions from gravity anomalies and an appropriate modeling [4, 5]. An important result from these studies is that cratonic areas (corresponding to the oldest parts of continents) are likely depleted in heavy minerals (in particular in minerals containing iron oxide), in agreement with the tectosphere hypothesis. These works were further applied to constrain chemical heterogeneities at a regional scale [9, 10], and to the determination of mantle viscosity profiles from tomographic models and gravity data [18]. I used a similar modeling to filter a collection of seismic and thermo-chemical models derived from Monte-Carlo (Metropolis) search with geoid observations. This method was applied to North-America [23] and to Australia [28].
• Radial distribution of seismic anisotropy in the crust and in the upper mantle. Seismic anisotropy is a powerful tool to map deformation within the Earth mantle and crust. Its stratification suggests that different fabrics are present at different depths, resulting from different past and present geodynamic events asthenospheric flow). I conducted several studies to recover the radial distribution of azimuthal seismic anisotropy of Rayleigh-wave in different regions. Beneath the central-eastern United States, these studies identified three distinct anisotropic layers related to different events [12, 13], the shallowest layer being related to past orogenies, and the deepest one being linked to current asthenospheric flow (Figure 1). Beneath the western Alps, anisotropy is also distributed in three layers, and the anisotrotopic pattern in the intermediate layer is consistent with slicing of the European lower crust [21]. Since I arrived at the IES, I collaborated to similar studies focusing on eastern China [30], eastern Tibet [40], and the Sea of Japan [43].
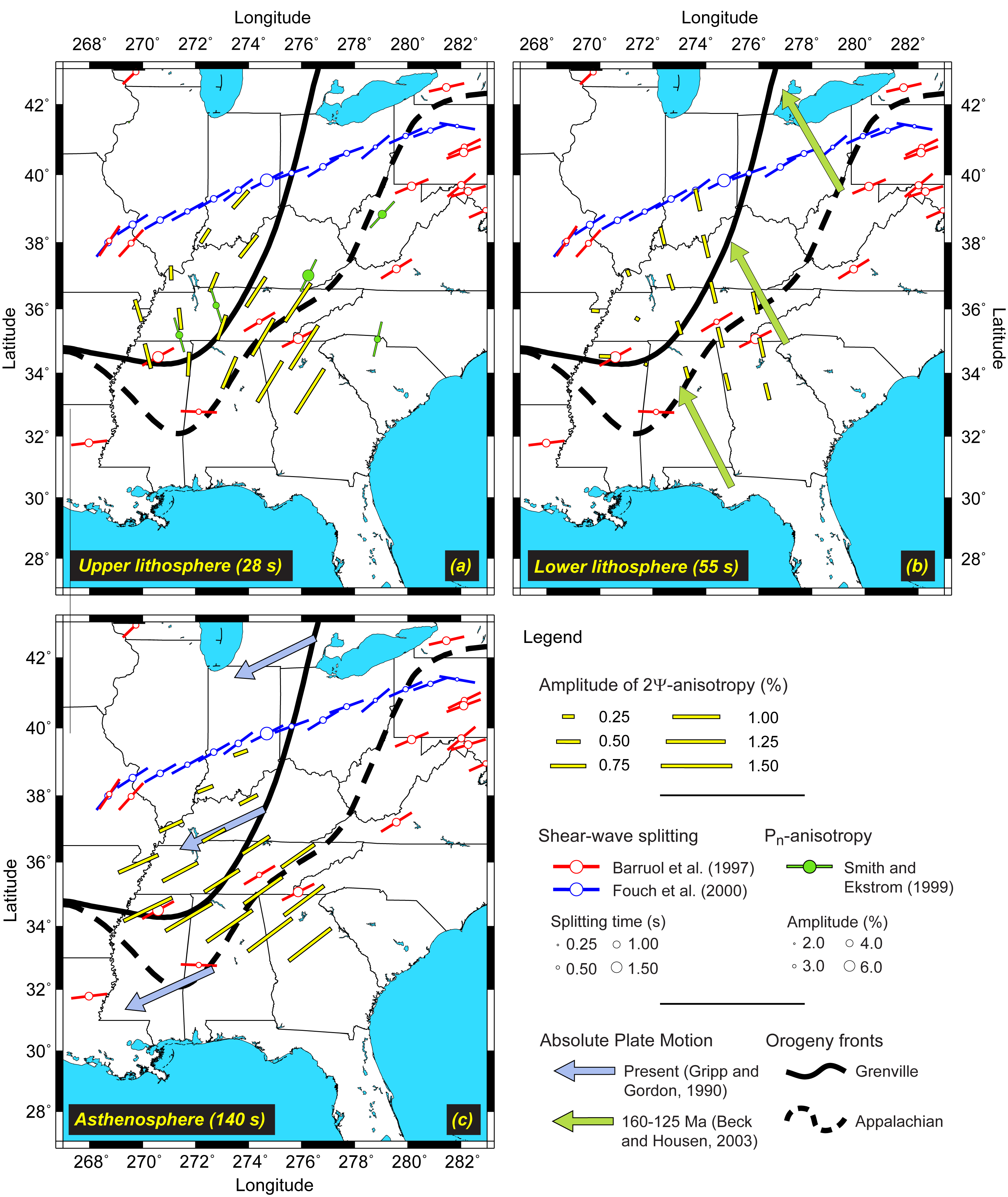
Figure 1. Distribution of Rayleigh wave azimuthal anisotropy beneath the East-Central U.S. at periods of 28 s, 55 s, and 120 s (Deschamps et al., 2008).
Structure and dynamics of the lower mantle
• Inferring the Earth's lower mantle thermo-chemical structure. Several seismological observations indicate that large-scale chemical heterogeneities are present in the lowermost (> 2500 km) mantle. The detailed nature of these heterogeneities is still a matter of debate. To better constrain the lower mantle thermo-chemical structure, I have developed quantitative methods that interpret lower mantle seismic tomography maps in terms of thermo-chemical structures [6, 7, 8], and test models of mantle dynamics against geophysical observables [11]. These studies clearly indicate that seismic tomography cannot be explained by purely thermal anomalies. Compositional anomalies should also be present, and normal mode data suggest that excess in iron in LLSVPs in a good candidate for such variations [8]. A key issue is to model as accurately as possible the equation of state of the mantle's mantle rock assemblage to determine the thermo-elastic properties of this assemblage at mantle temperatures and pressures, which allows, in turn, to estimate the sensitivities of seismic velocity and density to temperature and composition [6, 25]. This modelling shows that hot recycled oceanic crust (MORB) hardly explain LLSVPs, unless these MORB are much hotter than the surrounding mantle (by 1000 K and more). By contrast, it indicates that LLSVPs likely consist of regions enriched in iron oxides by a few percent and hotter by ~ 500 K, compared to average mantle (Figure 2).

Figure 2. Likelihoods of relative seismic velocity anomalies as a function of temperature anomaly for two possible composition (recycled oceanic crust in blue; and iron enriched material in red/orange) (Deschamps et al., 2012).
• The mode of convection of the Earth's mantle. The mode of convection of the Earth's mantle is closely related to its thermo-chemical structure. The detailed flow pattern is still debated, and several parameters may play a key role. An important goal of my research activities is to identify these parameters and estimate their influence. For this, I perform numerical simulations of convection with StagYY, a code developped by Paul Tackley and collaborators. Exploration of the model space of thermo-chemical convection in 3D-Cartesian [15, 16] and spherical [31, 35] geometries allowed the identification of parameters that are needed to maintain reservoirs of dense material in the deep mantle, the most sensitive parameter being the density contrast between the dense and regular material (measured by the buoyancy ratio). Viscosity variations with temperature and composition further plays a significant role. Models of thermo-chemical convection including moderate buoyancy ratio and large thermal viscosity contrast lead to the formation of reservoirs of dense material that can survive convection for periods of time comparable to the age of the Earth (as shown in Figure 3). Importantly, plumes are generated at the top of these reservoirs and entrain small fractions of dense material towards the surface with a rate of entrainment that is compatible with the geochemical signals observed in ocean island basalts (OIB) [24].

Figure 3. Snapshots of temperature (top row), composition (middle row), and post-perovskite (bottom row) caclulate by simulations of thermo-chemical convection (Deschamps et al., 2018).
At the base of the mantle, the post-perovskite (pPv) phase is also expected to influence the flow pattern. In collaboration with Yang Li (now at IGGCAS, Beijing), I have conducted series of simulations to better constrain these effects. Our calculations showed that the presence pPv with a very low viscosity does not substantially influence the shape and stability of the reservoirs of dense material [33]. They further pointed out that the core-mantle boundary (CMB) temperature and the slope of the pPv phase transition that best explain observations are in the range 3700-3800 K and 13-16 K, respectively [38]. Finally, additional simulations indicated that small patches of may be temporarily stable within reservoirs of dense material [41], which way in turn provide an explanation for the observed ultra-low seismic velocity zones (ULVZ).
• Additional constraints on the Earth's mantle deep structure. Seismic wave speeds anomalies alone cannot fully resolve the thermal and compositional signals from which they originate. This implies that additional constraints independent from seismic travel times are needed to infer the structure of the deep mantle with a good level of confidence. Normal mode data bring essential clues on density, but remain uncertain. In the past five years, I explored the influence of mantle structure on other type of constraints, including electrical conductivity, CMB dynamic topography, and CMB heat flux, and seismic attenuation in the deep mantle. I have also collaborated to studies that inferred thermal conductivities of mantle minerals at lower mantle pressures. These data allows reconstructing lower mantle thermal conductivities, and bring key information on the structure and dynamics of the lower mantle.
- Electrical conductivity. Using available electrical conductivity data for mantle minerals, I have calculated 3D distributions of electrical conductivity in the lower mantle for both purely thermal and thermo-chemical structures [34, 42]. Electrical conductivity distributions predicted by these two models strongly differ with one another, both in pattern and amplitude. In addition, the electromagnetic responses (C-responses) associated with each of these two end-member models are very different and may be used to infer the nature, purely thermal or thermo-chemical, of the lower mantle, provided that accurate long period data of magnetic field variations can be obtained.
- Core-mantle boundary dynamic topography. To assess whether core-mantle boundary (CMB) topography can bring constraints on the deep mantle structure, I have calculated CMB dynamic topography induced by mantle flow for different models of mantle convection [48]. Results show that in purely thermal models, plume clusters induce positive topography. By contrast, in thermo-chemical models with density contrast (ΔρC around 100 kg/m3 or more, reservoirs of dense material induce depression in CMB topography. In addition, the long-wavelength (spherical harmonic degrees up to l = 4) dynamic topography and seismic shear velocity anomalies are anti-correlated for purely thermal models, while they correlate for models with ΔρC ≥ 100 kg/m3. This potentially provides a test to infer the nature, thermal or thermo-chemical, of low shear-wave velocity provinces (LLVSP) observed by global tomographic images. In another study, I more specifically addressed the effect of the viscosity of the post-perovskite phase on deep mantle dynamics and CMB topography [57]. Results show that low viscosity post-perovskite size andstructures of reservoirs of primordial material, and the topography beneath downwellings.
- Core-mantle heat flux. Lateral variations of heat flux at the CMB is a key parameter of Earth's core dynamics and geodynamo models. In collaboration with Dr. Hagay Amit and Dr. Gaël Choblet University of Nantes, France), I have investigated the influence of non-thermal effects on CMB heat flux and related models of geodynamo [37]. Our results show that low-latitudes magnetic flux and kinetic energy contributions are more time-dependent in dynamo models obtained with heat flux reconstructed from probabilistic tomography (which include chemical anomalies), and thus can recover better the observed latitudinal distribution of geomagnetic flux on the CMB.
- Seismic attenuation. Seismic attenuation strongly depends on temperature and may thus be used as a proxy for this parameter. In collaboration with Dr. Kensuke Konishi (IES) and Prof. Nobuaki Fuji (IPG Paris), I performed studies to recover variations in shear-wave velocity (VS) and quality factor (Q) in the lowermost mantle from inversion of seismic waveform data. In particular, we obtained radial models of VS and Q in the depth range 2000-2800 km at two different locations, beneath the Northern and Western Pacific [44, 53]. At the Western Pacific (WP) location, sampling the western tip of the Pacific LLSVP and the Caroline plume, both VS and Q are substantially lower than PREM. We then showed that WP models cannot be explained by thermal anomalies alone, but require excess in iron of 3.5 to 4.5 % from the CMB up to 2600 km (Figure 4). The temperature anomalies, estimated from Q, are around 300-400 K close to the CMB, and 150 K at shallower depths. By contrast, NP models may have a purely thermal origin and can be explained by a temperature excess of about 50 K. Finally, we extended our study beneath the Western Pacific to 3D-models of VS and Q [59].
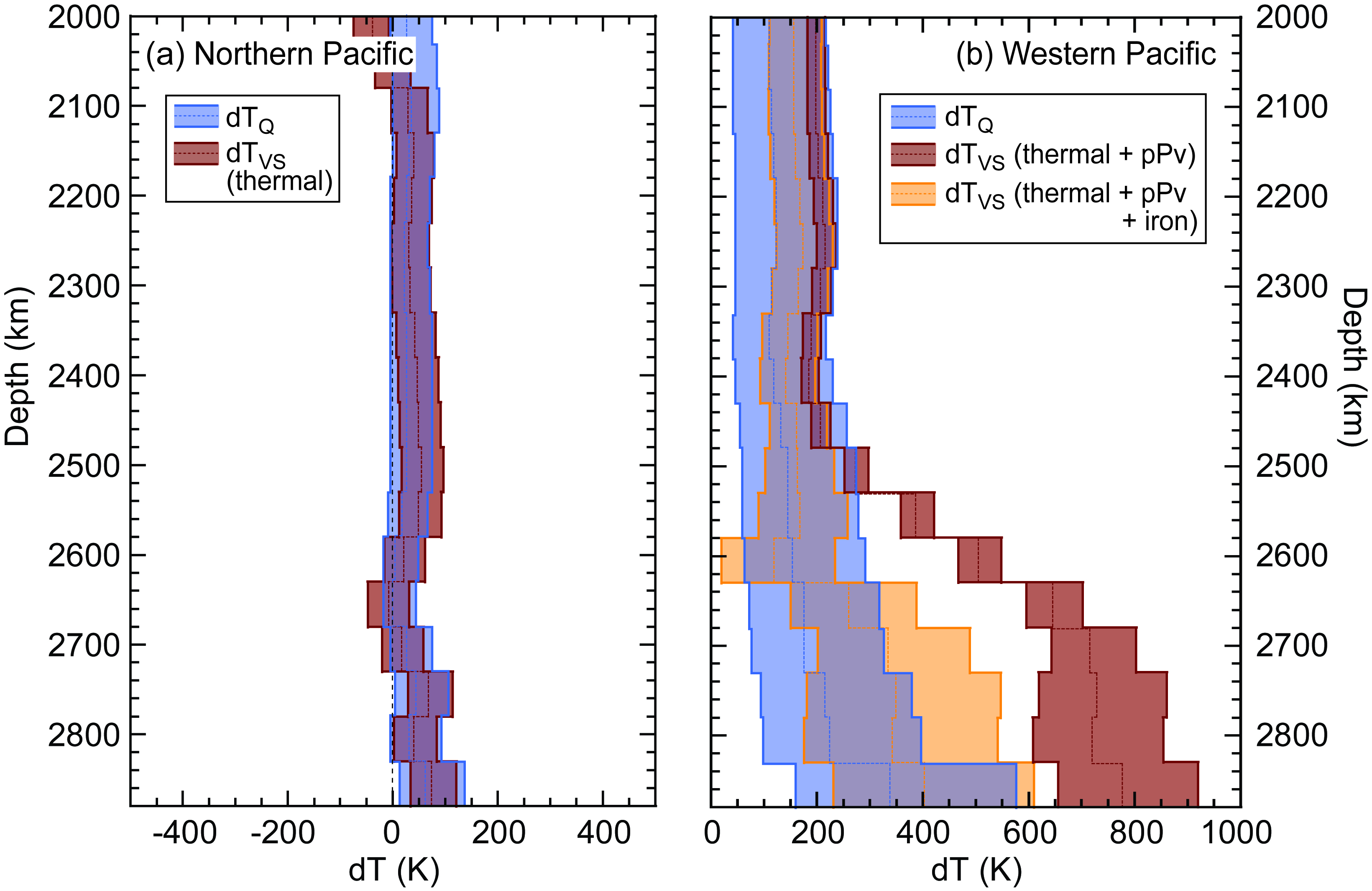
Figure 4. Vertical profiles of temperature anomalies inferred from anomalies in shear-wave speed (VS) and Q at two different location (left: Northern Pacific; right: Western Pacific) (Deschamps et al., 2019).
• Lower mantle thermal conductivity. In collaboration with Dr. Wen-Pin Hsieh, I modelled lower mantle thermal conductivity from high pressure mineral physics data. Our datasets [46, 49] and calculations show the thermal conductivity for a pyrolitic mantle at the bottom of the mantle and determined along an adiabat with potential temperature 2000 K is equal to 8.6 W/m/K. Using 3D thermo-chemical models from probabilistic tomography, which include variations in temperature, iron content, and bridgmanite fraction, we have also calculated maps of conductivity anomalies at the bottom of the mantle [54] (Figure 5). In regions known as large low shear-wave velocity provinces (LLSVPs), thermal conductivity is reduced by up to 26 % compared to average mantle, which may impact mantle dynamics in these regions. We then applied these results to model the lateral variations in heat flux at the core-mantle boundary (CMB).
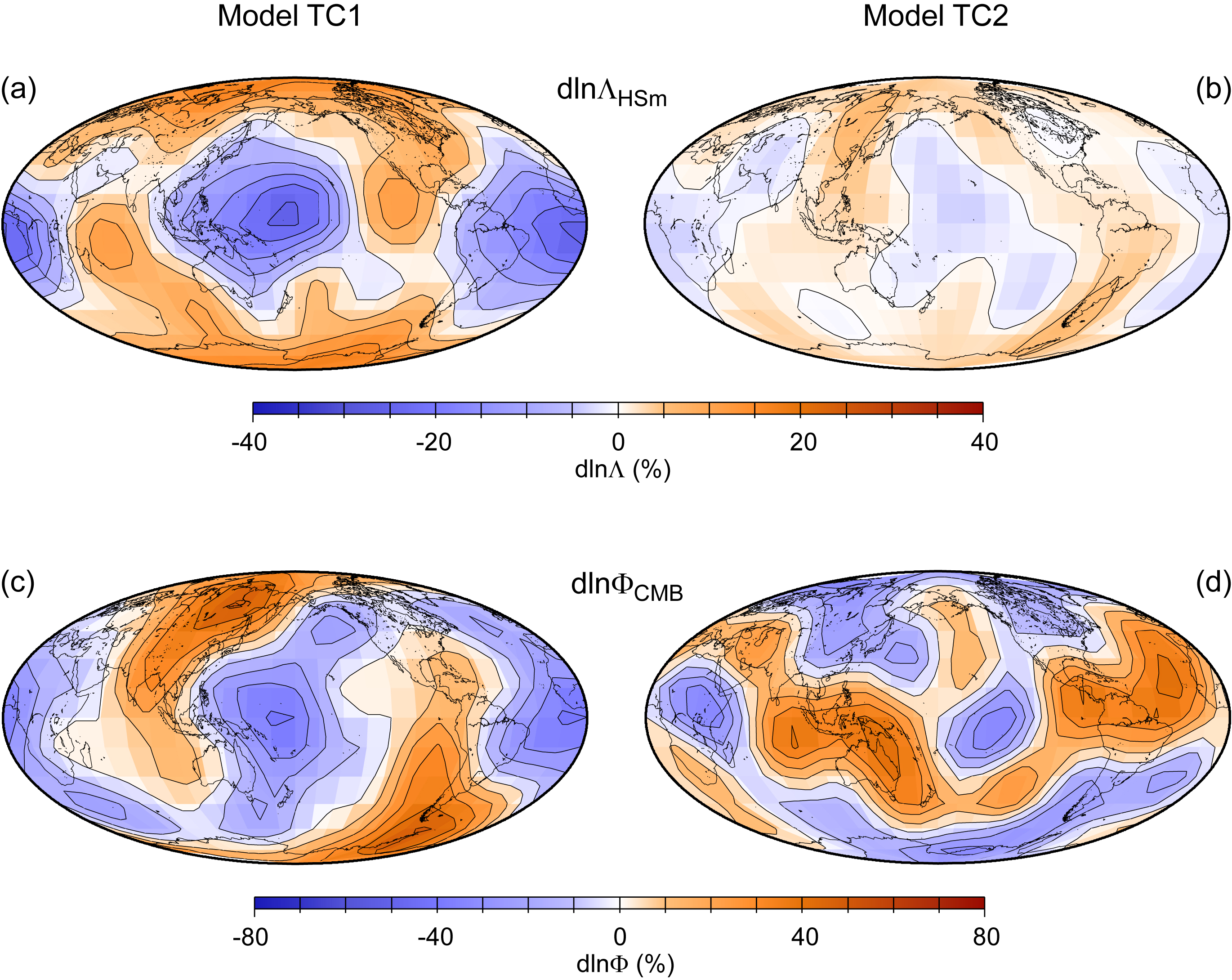
Figure 5. Thermal conductivity (top row) and CMB heat flux anomalies estimated from high pressure mineral physics data in [46] and [49], and two models of probabilistic tomography (left: Trampert et al., 2004; right: Mosca et al., 2012) (Deschamps and Hsieh, 2019).
Heat transfer and evolution of rocky mantles and ice shells
Like the Earth, other rocky planets and icy bodies are cooling down. Volcanism is the surface expression of this cooling, and exploration of the Solar System by automatic spacecrafts indicate that volcanic activity under various forms (e.g., water jets and cryo-volcanism in icy bodies) has affected or still affects most of the visited planets and satellites. Planetary cooling also involves heat transfer through mantle or ice shells, suggesting that thermal convection may operate or have operated in most planets and large or medium-size icy bodies.
In the case of outer shells of icy moons and dwarf planets, a key aspect to take into account is the fact that viscosity of ices strongly depends on the temperature. This lead convection to operate in the so-called stagnant-lid regime. In this mode of convection, a rigid and nearly immobile layer forms at the top of the system, and the flow is confined beneath this rigid layer. Furthermore, within this lid heat is transported by conduction, which strongly reduces the heat transfer and impacts the thermal evolution of these objects.
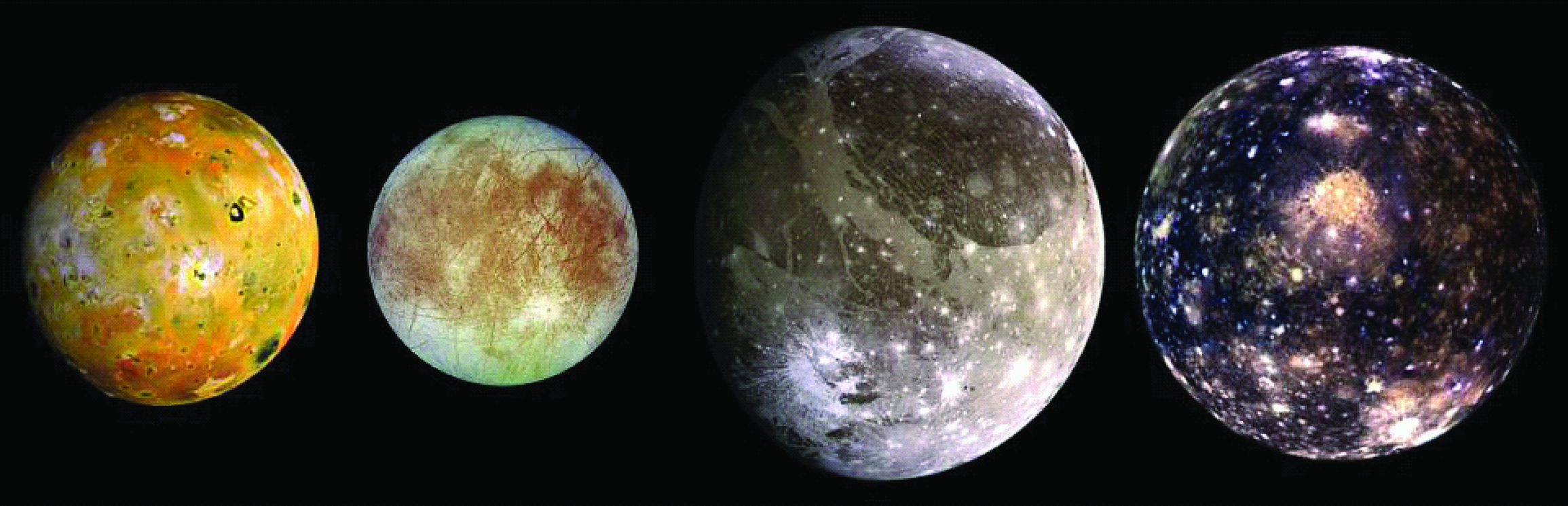
Io, Europa, Ganymede and Calisto seen by the spacecraft Galileo (© NASA).
• Scaling relationships for stagnant lid convection and thermal evolution of icy bodies. During my PhD, and then in ETH Zürich and at the IES, I have conducted series of numerical experiments of thermal convection to better constrain the heat transfer through the silicate mantles of rocky planets, and through the outer ice shells of icy moons of giant planets. From these experiments, I determined scaling laws for heat flux in the case of stagnant lid convection in 2D-Cartesian geometry [2], which I have applied to model the radial structure, dynamics, and evolution of the outer ice layer of the large moons of giant planets [1, 3, 22]. An important conclusion of these applications is that the presence of impurities in the sub-surface oceans, such as ammonia (NH3) and methanol (CH3OH), act as anti-freeze compounds that are able to delay or even stop the crystallization of the ocean, thus leaving a sub-surface ocean. Simulations in 3D-Cartesian [29] (Figure 6) and spherical [32] geometries allowed to build refined scaling laws and to model thermal evolutions with a better accuracy. I have recently started calculations to determine the influence of internal heating (i.e., the release of heat due to tidal dissipation within the ice layer) and of temperature-dependent thermal conductivity on stagnant lid thermal convection.

Figure 6. Snapshops of two simulation with stagnant lid convection in 3D-Cartesian geometry (Deschamps and Lin, 2014).
Cryo-volcanism implies that pockets of liquids should be present within the outer ice shells of icy bodies. Models of convection help to identify and better constrain the parameters leading to the generation of melts in systems that include both bottom heating and heterogeneous internal heating. With Kenny Vilella (currently at the University of Hokkaido), I studied more in details the role played by heterogeneous heating related to dissipation and distributed as a function of viscosity [60]. For this, we run series of numerical experiments in which the distribution of heating depends on local viscosity. We applied our results to the generation of melting within Europa. Our main finding is that melting can be generated fairly close to the surface (~ 5 km) as long as the ice layer remains thin (< 40 km).
• Mechanisms driving convection. Systematic studies are essential activities to obtain a better understanding of the fundamental mechanisms driving convection and the role played by specific parameters, for instance the mode of heating, basal or internal. For purely basal heating, simulations show that the average temperature and the heat transfer decrease as the curvature increases. Calculations with mixed heating further indicate that hot plumes become less vigorous and that the average temperature increases as the rate of internal heating increases [19] (Figure 7). In the case of purely volumetrically heated spherical shells, numerical simulations show that the mechanism controlling the convection in Cartesian geometry (which consist in the conductive thickening of a fluid layer below the surface until instabilities occur) is also valid in spherical geometry, and that it is independent of the curvature of the shell [26].
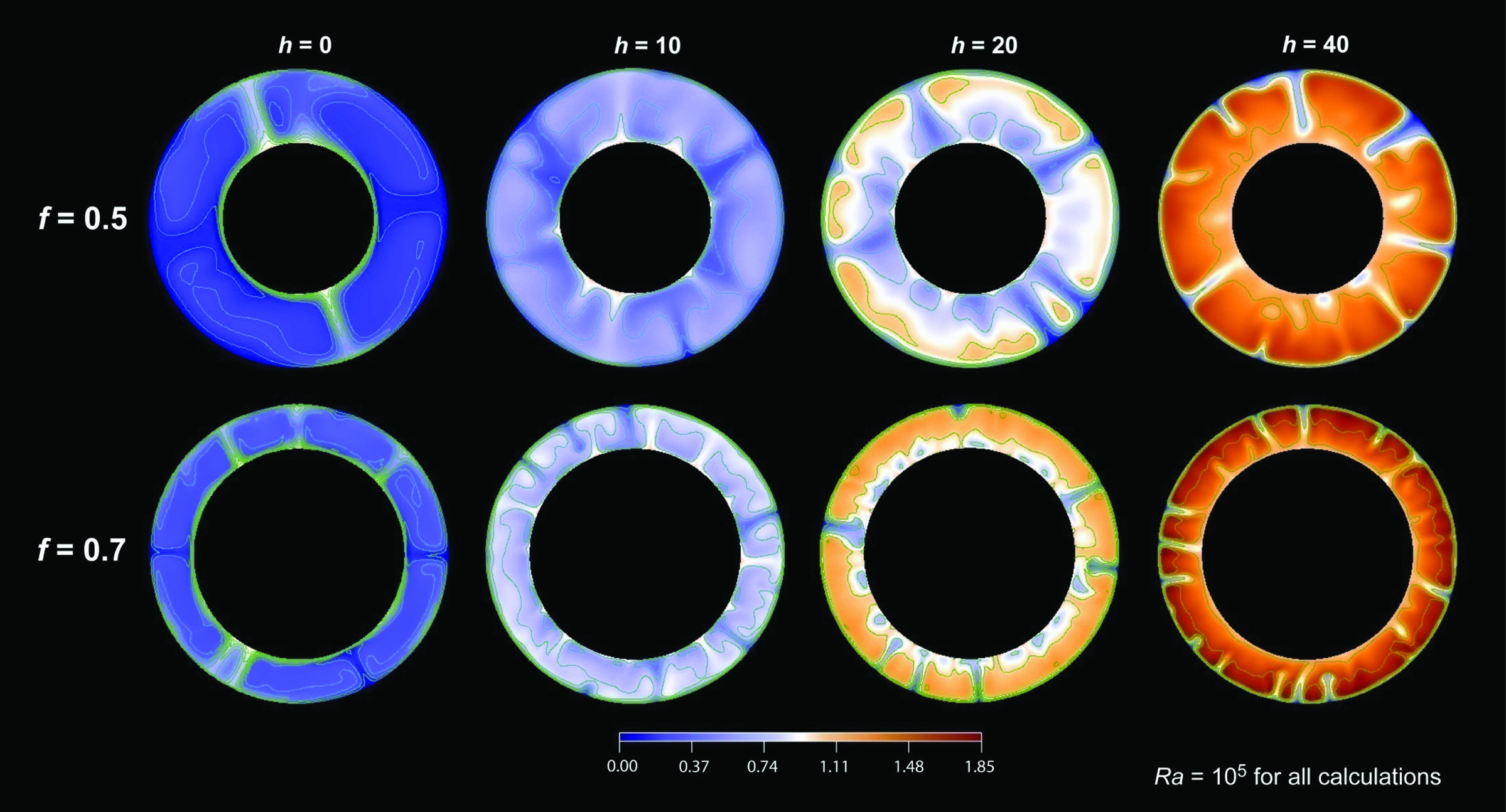
Figure 7. Effect of internal heating on temperature. Internal heating (H) increases from left to right, and two core size are shown (f = 0.55 and f = 0.70) (Deschamps et al., 2010).
Still in the case of mixed heating, and in collaboration with Kenny Vilella, I built theoretical scaling laws from 2D and 3D-Cartesian simulations [50]. These scalings successfully predict the thermal structures of systems with large internal heating rates, but fails at describing purely basal heating (Rayleigh-Bénard systems. The differences between moderate and large internal heating rates are interpreted as differences in the mechanisms generating thermal instabilities. Three different mechanisms are potentially involved: conductive growth of the TBL, TBL erosion, and mechanical interaction, the last two being present only for moderate internal heating rates, in which hot plumes are generated at the bottom of the system and are able to reach the surface.
• Sputnik Planitia. During its flyby of Pluto, in July 2015, the NASA's spacecraft New Horizon revealed that the surface of this dwarf planet is geologically very complex. Among the most interesting features is a nitrogen ice glacier, Sputnik Planitia, whose surface is split in a network of polygons of ~ 20-40 km in size, a structure which is characteristic of the dynamic topography induced by convection. In collaboration with Kenny Vilella, I have investigated the dynamics of Sputnik Planitia using 3D-Cartesian simulations of thermal convection. An important conclusion of our study is that the polygonal patterns observed at the surface of Pluto are inconsistent with bottom heated convection, as previously thought, but may instead result from volumetrically heated convection [45]. In this later case, convection may be driven by successive phases of heating and cooling of the glacier triggered by long term variations of Pluto's orbital parameters. More precisely, as Pluto's surface temperature increases Sputnik Planitia heats up by conduction and remains dynamically stable. As surface temperature starts decreasing, the glacier releases the heat it stored during the heating phase and becomes unstable, i.e. its cooling is operated by convection.
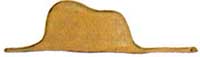